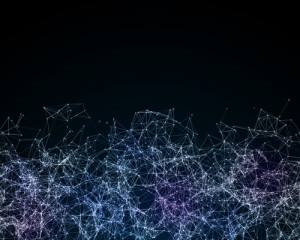
Nuclear Power and Nanomaterials: Big Potential for Small Particles
Nuclear power plants are large, complex, and expensive facilities. They provide approximately 19 percent of U.S. electricity power supply,1 and in the process consume enormous quantities of water. However, a class of very small particles may be gearing up to lend a helping hand in making power plants more efficient and less costly to operate. This article will briefly introduce nanomaterials and discuss ways in which some of these particles may make nuclear power plants more efficient.
The race to synthesize, engineer, test, and apply new nanoscale materials for solving difficult problems in energy and defense is in full swing. The past twenty five years have ushered in an era of nanomaterials and nanoparticles – objects with at least one dimension between 1 and 100 nanometers2 – and researchers are now implementing these materials in areas as disparate as neuroscience and environmental remediation. To provide a sense of scale, most viruses are a few hundred nanometers in size, most bacteria are a few thousand nanometers in size, and a period at the end of a sentence is about a million nanometers. This new category of materials has ignited the imaginations of scientists and engineers who envision nanomaterials capable of tackling difficult problems in energy, healthcare, and electronics.
Nanomaterials are not new, and indeed occur naturally all over Earth. This includes viruses, the coatings of a lotus leaf, the bottom of a gecko’s foot, and some finely powdered clays. These objects represent natural materials with significant, and often highly functional, nanoscale features. Some researchers have even discovered signs of nanoscale materials in space.3 One of the oldest documented applications of nanomaterials dates back to the Lycurgus Cup, a 4th century Roman glass which was made out of a glass containing gold and silver particles. The result is a glass that appears green when lit from the outside, but red when lit from the inside.4 The effect results from the glass filtering various wavelengths of light differently depending on the various lighting conditions. Of course, the Romans did not know they were using nanoparticles in the process of making this glass.
But what makes nanoparticles interesting or unique? The answer to this question depends on the specific material and application, but a few themes persist. Because of their small size, the physical principles governing how particles behave and interact with their environments change. Some of these changes are due to how basic properties such as volume and surface area change as an object becomes smaller. As a sphere shrinks, the ratio of the surface area to the volume grows. This has far reaching implications for how particles interact with light, heat, and other particles. Visionary researchers are now looking into ways in which these interesting properties may make nuclear power plants more efficient.
One important implication for our discussion is the flow of thermal energy. Consider the process of transferring the thermal energy of your body from your hands to an ice cube. Clearly, you are (hopefully!) warmer than the ice cube. If you place the ice cube on a chilled dish and touch the ice cube with one finger, the cube will melt, but probably fairly slowly. Placing your entire hand over the top half of the ice cube increases the melting rate, and placing the ice cube in your hand and closing your fist further increases the melting rate. This is an example of thermal energy transfer via conduction. Conductive heat transfer from one object to another depends on the area over which the thermal transfer takes place. A larger contact surface area leads to faster conduction. But how does this relate to nanoparticles? As a particle becomes very small, the ratio of the particle’s surface area to its volume increases very rapidly. Since thermal conduction through volume is a function of surface area, particles with large ratios of surface area to volume are able to change temperature very quickly. If you place a large quantity of small cold particles in a warm body of water, the particles will heat quickly. If you take the same volume of particles, but instead compress it into one large particle, then that large particle will warm slowly. As this surface area to volume ratio increases with decreasing size, a general trend is for smaller particles to transfer heat more effectively than larger particles.
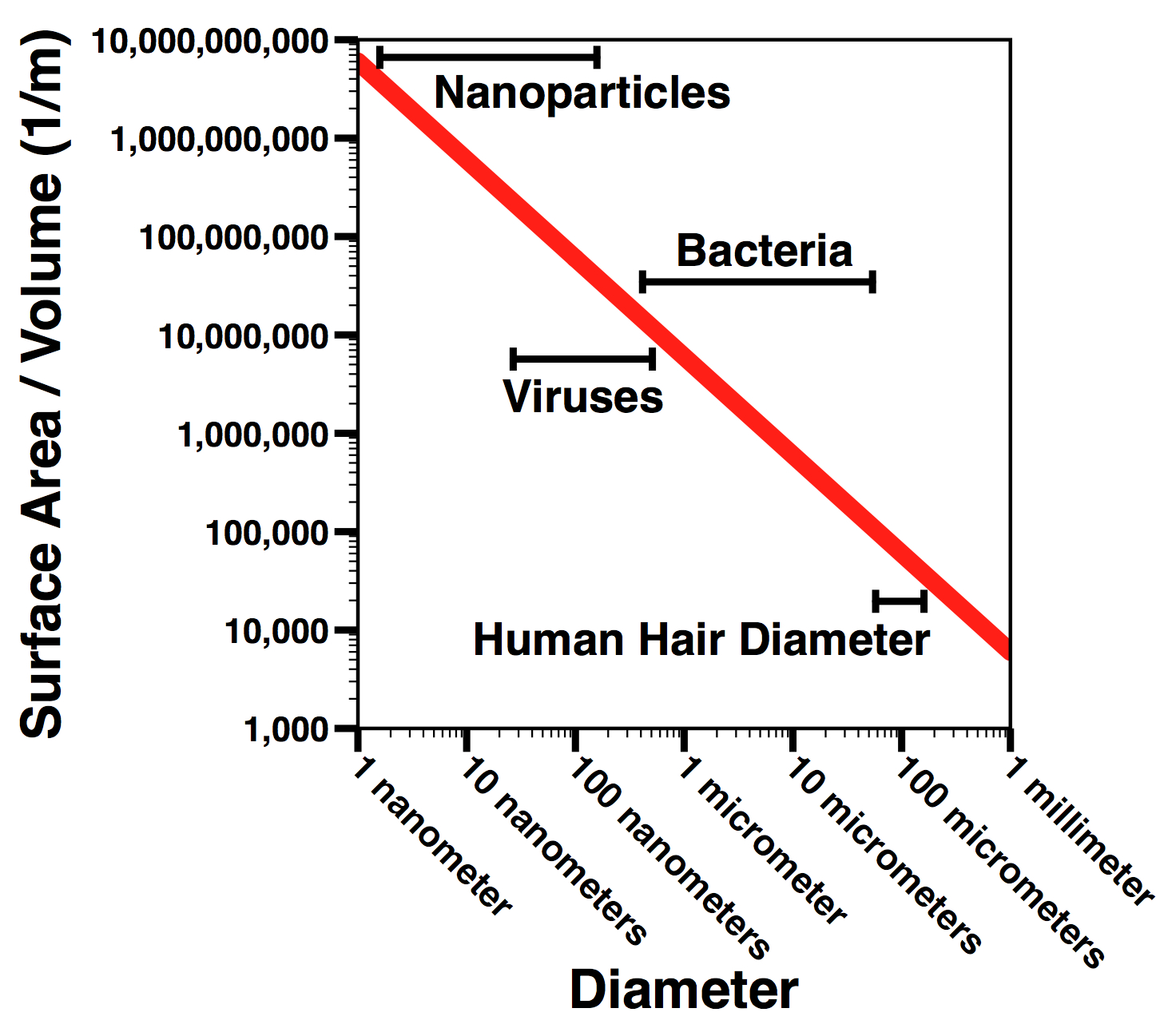
So how does this relate to nuclear power plants? Nuclear power plants are water-intense operations and rely on conductive heat transfer to convert nuclear energy to grid-ready electricity. The most common Western reactors are pressurized water reactors (PWRs) in which water is heated by pumping it through the reactor core, then pumping the hot water to a steam generator. This water flows through piping called the primary system and is kept in the liquid state by applying very high pressure through a device called a pressurizer. In the steam generator this primary system water transfers much of its heat to water in a secondary system. High-strength piping, which is a very effective heat conductor, keeps the water in the two systems from directly contacting each other. The secondary system’s water turns to steam when it absorbs the heat from the primary system. The steam is then directed via piping to drive a turbine, which turns an electric generator, thus completing the cycle of converting nuclear energy to readily usable electricity for the grid. After passing through the turbines, the steam is captured and condensed for recycling. This reclaimed water can then be sent back through the steam generator. However, a significant amount of the energy of this steam is lost to the atmosphere via a third system of cooling water that is used to condense the steam. Large amounts of water (in the form of water vapor) are released to the environment in this process. Think of the water vapor plume at the top of the iconic cooling towers seen in the cartoon TV show The Simpsons. (Not all nuclear power plants use these types of cooling towers, but all must emit heat to the environment through some means of cooling.)
A new class of nanomaterials called core-shell phase change nanoparticles may help in reducing the water loss. First, let’s parse the name of the nanoparticle. The core-shell nomenclature refers to the fact that the particle has a center made out of one material, and an outer skin made out of another material. The phase change component of the name refers to the fact that the particle center changes from a liquid to a solid under certain conditions. These particles may be mixed into the water used for transporting the thermal energy generated within the reactor. Once mixed into the reactor water, the particle cores melt as the water picks up thermal energy from the reactor. The melted material in the particle core is contained by a shell, which remains solid at reactor temperatures. Thus, as the water leaves the reactor it carries with it tiny particles containing bundles of liquid thermal energy wrapped in a solid core. The notion is that as these particles travel to the cooling tower, they solidify and dissipate their heat into the surrounding water, thus decreasing the amount of water needed to convert the thermal energy created by the reactor to steam for turning turbines. Additionally, since these particles do not vaporize, they are much more easily retained for recycling. The Electric Power Research Institute is currently working with scientists at Argonne National Laboratory to commercialize these particles and has suggested that this technology could decrease power plant water requirements by as much as 20 percent.5
Another nanoparticle-based approach for increasing reactor efficiency seeks to tackle a different problem. Pressurized water reactors place the water in direct contact with the fuel rods of the nuclear reactor. However, bubbles that form on the surfaces of the fuel rods can significantly decrease efficiency by insulating the rods from the water. When this happens, heat transfer efficiency suffers. One lab at the Massachusetts Institute of Technology (MIT) has implemented alumina nanoparticles that coat the fuel rods and prevent the buildup of bubbles on the heating elements. Alumina, a compound of aluminum and oxygen, is stable and has a high melting temperature. Testing these particles in the MIT reactor, the group found that the alumina nanoparticles coated the fuel rods. The result was an increase in the efficacy of the reactor. The engineers explain the findings by suggesting that the alumina nanoparticles allow for quick removal of the bubbles forming on fuel rod surfaces, thus minimizing the insulating layer of bubbles and maximizing heat transfer efficiency.6 To validate this, the researchers heated identical thin, steel wires a fraction of a millimeter in diameter. One wire was submerged in water, the other in a nanofluid containing alumina particles. The wires were heated to the point of boiling the surrounding fluid. After boiling the wires were examined using a powerful electron microscope. The experimenters observed that the wire heated in the nanofluid was indeed coated with nanoparticles, while the other wire maintained its original smooth surface.
Most importantly, there are also potential safety applications of having nanofluids capable of quickly transporting large quantities of thermal energy. One proposal calls for the use of nanofluids in standby coolant stored in Emergency Core Cooling Systems (ECCS). The ECCS are independent, standby systems designed to safely shut down a reactor in the case of an accident or malfunction. One ECCS component is a set of pumps and backup coolant to be sprayed directly onto reactor rods. Such systems are critical in preventing a loss of coolant accident (LOCA) from spiraling out of control. Because ECCS have backup reservoirs of coolant, technologies that make this backup coolant more effective at removing heat from the reactor could improve the safety of reactors. Because nanofluids can increase the heat transfer efficacy of water by 50 percent or more, some researchers have suggested that they may also be useful in emergency scenarios.7
Steam generators at both nuclear and coal power plants accounts for approximately 3 percent of overall freshwater consumption in the United States. Generally speaking, nuclear power plants consume about 400 gallons of water per megawatt-hour (MWh). Their coal and natural gas counterparts consume approximately 300 and 100 gallons per MWh, respectively.8 Thus, nuclear power plants stand to gain considerably by becoming more water-efficient.
However, there are many hurdles to tackle before nanoparticles can be safely and effectively used in operating power plants. Scaling up particle production to the large volumes of particles necessary for implementation in a power plant is expensive and labor intensive. New synthesis infrastructures may be necessary for large-scale production of these tiny particles. Additionally, broad adoption of this technology will not occur until significant cost savings are proven effective at a functioning plant. As a result, particles must be made available at a cost reasonable for adoption by power plant operators. A rough cost estimate can be made using commercially available alumina nanoparticles, as these particles have been tested extensively in the heat transfer literature. A typical nuclear power plant in the United States supplies enough electricity to power 740,000 homes. To do this, the plant requires between 13 and 23 gallons of water per home per day. Thus, water usages for the plant may range from 10 to 17 million gallons per day. Current vendors of aluminum oxide nanoparticles sell 1kg of nanopowder for around $200. With an expectation that economies of scale would bring that price down to $100/kg and that the particles could be easily recovered and recycled, loading a nuclear power plant with a 0.1% volume fraction of alumina nanoparticles would cost about $14.7 to $25 million per power plant. This is a substantial initial investment. Naturally, if nanoparticles were to cost $10/kg, then particle outfitting costs of $1.5 to $2.5 million per nuclear plant could be achieved. If 100 percent recovery of the particles could be achieved, then this initial cost would be recovered over time by the expected 2 to 4 percent increase in plant efficiency.
In addition to cost-benefit analysis, extensive testing must be performed to ensure long-term application of these particles does not threaten the operational safety of the plant. To accomplish this, smaller scale reactors (like those housed at research facilities and universities) may test these particles over the course of years to track the impacts of long-term use. Potential pitfalls include increased corrosion, system clogging, and nanoparticle leakage into wastewater. Corrosion engineers will be needed to validate the degree to which nanoparticles contribute to the overall aging of reactors in which they are used. Nanoparticle designers and hydrodynamicists will be needed to ensure that system clogging is manageable. Additionally, filtration experts and the Environmental Protection Agency will be needed to establish best practices for minimizing the amount of nanomaterial that exits the facility, as well as understanding and quantifying the environmental impacts of that emitted material. None of these potential roadblocks are trivial. However, while the challenges seem large, it is encouraging to see potential applications of nanotechnology in power plants.
To fight the climate crises, we must do more than connect power plants to the grid: we need new policy frameworks and expanded coalitions to facilitate the rapid transformation of the electricity system.
The stakes are high: how we manage this convergence will influence not only the pace of technological innovation but also the equity and sustainability of our energy future.
The Federation of American Scientists supports the Critical Materials Future Act and the Unearth Innovation Act.
The Trump administration has an opportunity to supercharge American energy dominance through MESC, but they must come together with Congressional leaders to permanently establish MESC and its mission.