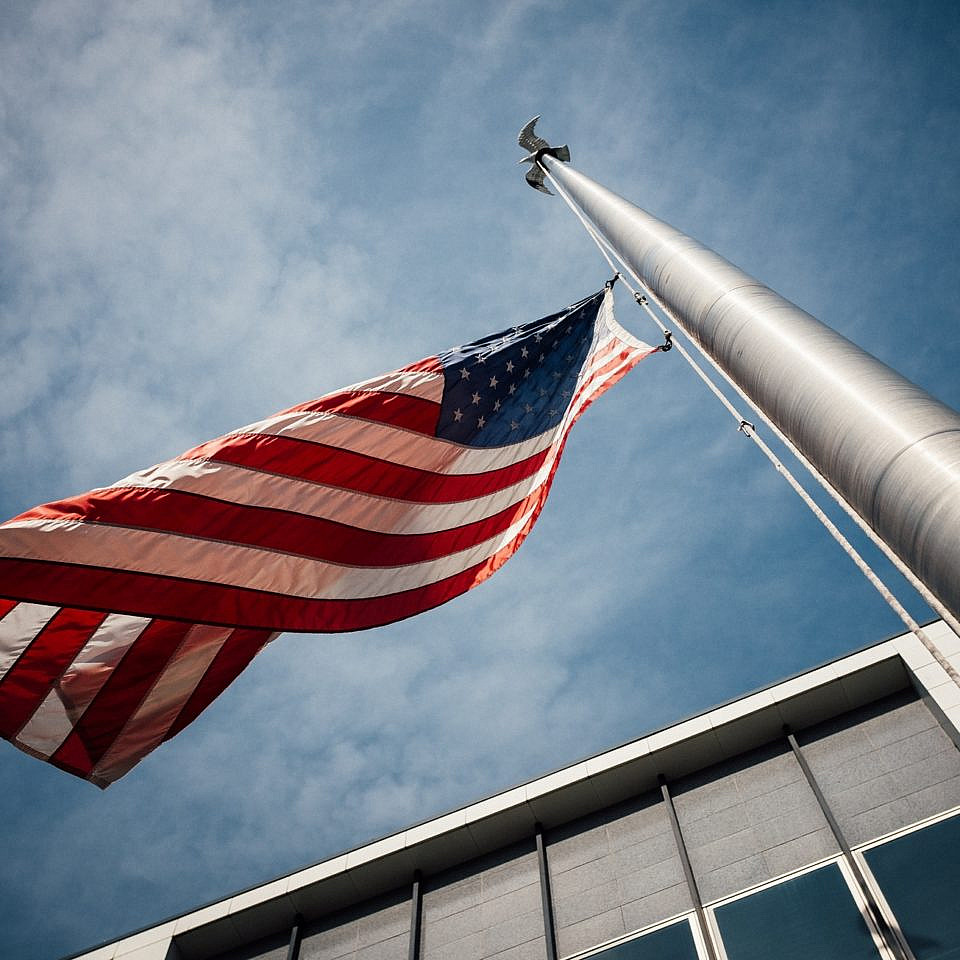
Public Interest Reports Archive
Spring 2005
This issue featured on the passing of FAS founder and Manhattan Project veteran Philip Morrison, the FAS building technology program, the future of DOE labs, and the Virtual Patient.
Summer 2005
This issue highlighted the upcoming 60th anniversary of the founding of FAS, the Reliable Replacement Warhead, the ineffectiven nuclear bunker buster, and the regulation of virus research.
Winter 2005
This issue featured Budget Priorities for 2006, conventional weapons displacing nuclear weapons, a survey of first responder training, and the nonproliferation case for maintaining the EU arms embargo on China.
Spring 2007
This issue highlighted the FAS presentations at the 2007 AAAS Annual Meeting in San Francisco, CA. Articles included Richard Garwin’s talk on plutonium recycling, the global nuclear energy pratnership, responses to a dirty bomb attack, and using advanced learning technologies to revolutinize education.
Summer 2007
New building technologies and the FAS demonstration houses are featured in this issue. Articles included an update on the FAS Building Technology Project, a recount of the demonstration project and meetings in Turkey and Azerbaijan, and the FAS response to Hurricane Katrina.
Fall 2007
This issue focused on learning technologies topics including virtual worlds, the Immune Attack game, online tools, and an update on the status of the Higher Education Act.
Winter 2007
This issue focused on the Small Arms trade with articles on how to stem the trade of illicit small arms, recent United Nations action on small arms, Venezuela’s challenge to control state ammunition stockpiles, and illicit trade in Africa.
Spring 2008
This issue features the updated FAS report Toward True Security, the diversion of small arms, a failed intelligence satellite, and an Oped by Arthur Obermayer.
Fall 2008
This issue highlights the FAS awards programs, the FAS panel discussion on the U.S. and China’s Energy Policies, the FAS panel discussion on U.S. Nuclear Policy for the 21st Century, and the National Center in Advanced Information and Digital Technologies.
Spring 2009
Articles included high performance buildings, ways to improve residential energy efficiency, structurally insulated panels, how to modernize the DOE weatherization assistance program, and FAS in China as it rebuilds after the 2008 earthquake.
Summer 2009
Articles include dual-use research case studies, a new nuclear policy to eliminate nuclear weapons, the 64th anniversary of the bombing of Hiroshima, the START follow on treaty, securing Venezuela’s arsenals, and a new FAS study on scientists and law enforcement.
Spring 2010
Read the first message by new FAS President Charles D. Ferguson. Other articles covered the development of Immune Attack, creating a pilot program in a virtual world to train building inspectors, and the diversion and theft of small arms in Iraq.
Summer 2010
This issue reported on the new Nuclear Posture Review, the New START Treaty, the Nuclear Non-Proliferation Treaty Review Conference in NYC, the premier of the FAS video “Paths to Zero”, and newly elected FAS Board Officers and Board Members.
Fall 2010
This issue featured articles on a systems resilience and nonproliferation, an analysis of non-state actors and nuclear control, cyber warfare, a systems approach to Yemen’s environmental issues, and much more.
Winter 2010
This issue of the FAS Public Interest Report featured the FAS Biosecurity Program, nuclear energy programs in China and France, and questions of nuclear deterrence.
Spring 2011
FAS launches a new design for the PIR. This issue features articles on illegal exports, nuclear power, detecting radiation at borders, and the next steps for U.S. and Russia nuclear arms reductions.
Summer 2011
This issue focuses on energy. Articles include protecting nuclear installations from disaster, carbon capture and storage systems, safety and oversight of U.S. waters, lessons learned from the BP oil spill, the impacts of biofuels on climate change, and more.
Fall 2011
This issue covers chemical, biological, nuclear, and radiological topics. Articles include security at U.S. chemical facilities, biological agents in the laboratory, the 2011 BWC, dirty bombs, the Nuclear Security Summit in Seoul, infectious dieases, the risks posed by radioactive materials, and more.
Winter 2011
This issue features articles on space security. Articles include new UN measures to build transparency, road rules for space, managing risk in space, the phased adaptive approach missile defense system, bringing Twitter to North Korea, and more.