Regulations, funding, and knowledge gaps: Challenges and opportunities in bringing agricultural biotechnology to market
Innovations in agriculture will play an increasingly important role in America’s quest to ensure resilient and sustainable production of food, medicine, and bioenergy products. Biotechnology, spurred by advances such as cheap sequencing, offers a realm of possibilities for novel agricultural inputs, such as more targeted pesticides that are less toxic and less likely to cause tolerance, less carbon-intensive alternatives to fertilizers, and more climate-resilient crop varieties.
However, research and development of new agricultural biotech products can be expensive and time-consuming, due to the large physical scale and long timelines of field trials. At the same time, federal funding for agriculture research has historically paled in comparison to funding for defense, energy, and human health. For example, in 2022, the NIH’s R&D budget was more than 16 times that of the USDA’s.
The Biden administration has demonstrated its recognition of the need to accelerate research and development in agricultural biotechnology, featuring it prominently in 2022’s Executive Order on Advancing Biotechnology and Biomanufacturing Innovation for a Sustainable, Safe, and Secure American Bioeconomy. Additionally, a bill to expand authorization of funding for a moonshot USDA research grant program, AgARDA (Agriculture Advanced Research and Development Authority), has broad bipartisan support. At the same time, there has been a commensurate increase in private funding.
While this multi-front surge in enthusiasm and investment is welcome, many challenges remain in translating money, ideas, and laboratory results to the field and the market, including communication between the various stakeholders in agricultural biotechnology R&D. To better understand industry priorities and potential barriers to progress, we spoke to members of the executive team of Fall Line Capital (FLC), a venture capital (VC) and private equity firm that invests in food/agriculture startups. Fall Line’s investments include new biopesticides (Greenlight, Micropep), functional microbes (Pluton, Wild Microbes), and new equipment (Guardian Agriculture, Rantizo, LUMO), in addition to managing a farmland portfolio. As lifelong farmers as well as agriculture technology (agtech) investors, Clay Mitchell and Scott Day offer a multifaceted perspective on the current landscape.
We then outline actions for government actors that can address the challenges identified in our interview, in three key areas: regulatory oversight, federal R&D funding, and bioliteracy.
Q: What can the U.S. government do to provide a supportive landscape for new agricultural biotechnology?
Fall Line Capital: I think the biggest hurdles are regulatory. If the government wants to be truly supportive and innovative, it should be working to revamp the convoluted regulatory environment. The current system wasn’t designed to handle all the new technology being developed with novel mechanisms of action, so hurdles to creating and commercializing stifle innovation even more than they did in the past.
Looking at new technology like RNA– and micropeptide-based pesticides, it’s been a difficult process to get those products registered, even though they should be embraced: compared to conventional pesticides, they have the potential to be highly specific to the target organism and minimally toxic to non-target organisms. During GreenLight’s discussions with the EPA to register their RNAi biopesticide for tackling invasive potato beetles, the EPA seemed to understand that this sort of technology is the future, but movement through the registration approval process was slow nevertheless; the application sat there for five 5 years. There were dozens of other biological pesticide product applications, and the EPA had to give every application the same level of scrutiny, even if many were obviously ineffective. There’s pressure to register more biological products as a prominent alternative to traditional chemical products, despite generally low efficacy. This clutters up the process, and the EPA was already short-staffed after extensive attrition during COVID.
A substantial amount of the innovation is coming from small companies like Greenlight that don’t have the resources (which many of the large incumbent ag companies have) to navigate the current registration programs and protocols, which are spread across multiple agencies involved in regulating biotechnology: the USDA, EPA, and FDA. There needs to be a new concierge resource beyond what the Unified Website for Biotechnology Regulation currently provides, that could direct you to the right office, the right registration process, as well as appropriate funding opportunities and legal resources.
Q: What do you think are currently the most pressing challenges in agriculture?
FLC: Pest resistance continues to be a very serious concern in agriculture, so new and effective control measures need to be continually developed for all pests: weeds, insects, disease. There are two major pests of concern for my own farm in Western Canada. First, herbicide-resistant kochia weed, which has become a huge problem in the last five years all across the world. No one’s sure how exactly it spread so quickly. Second, flea beetles are decimating cruciferous crops. RNAi-based insecticides could be very effective here, if we can achieve sufficient persistence of the insecticide and avoid impacting non-target species.
In terms of challenges to agriculture-based businesses, there’s a lack of funding right now for getting tech to market. Funding for agtech from VC firms fell last year, as it did for most forms of tech. This was following a very strong period of agtech funding for the previous two years, during which we saw over-investment in several sectors, such as alternative meat and indoor farming. At the same time, agtech companies typically have long timelines to product launch and need more funding than just one VC can provide. Right now, many companies who come to Fall Line for money are just looking for short-term “bridge funding” so they can make payroll and buy time until they can demonstrate enough progress to raise a successful “series” round with good valuation and favorable investment terms. And no one is going public right now.
Q: What are common knowledge gaps for agtech startups regarding farmers’ needs?
FLC: Agtech startups are often centered around a great idea or technology that’s looking for a problem to solve — but it’s hard for a specific technology to meet the needs of a variable problem. Farmers’ needs and priorities (e.g. pests, nutrients, etc.) are incredibly diverse, varying dramatically by crop and location, even from one field to the next on the same farm, or even within the same field. Today, it is very hard to get an accurate understanding of what is needed or desired at the farm level because there is no easy way to connect with growers on a broad scale. Farm papers have diminished in popularity just like mainstream papers, radio has diminished as well. Unless you have the email address or cell phone number of a farmer, it is hard to connect directly with them now, and most farmers don’t like doing surveys of any type anyway — and those that do aren’t that representative of the industry. I think this is why most types of polls are becoming less accurate as it is increasingly more difficult to get a representative sample of opinions.
Farmers can be hesitant to adopt new technologies, since the risk can be high. And once they’ve been burned once by a product that failed to work as advertised, they’re unlikely to be willing to trust that company, or even that type of product, in the future. For example, last year, North Dakota State University scientists coordinated a large-scale field trial where it showed in a large field trial that most new biological products aimed at improving nitrogen-fixation in non-legume crops were ineffective at increasing yield. In general, the efficacy of biologicals can vary greatly depending on the exact field conditions, making it hard to reliably achieve the advertised result. There’s a huge jump from greenhouse results to field trials, another huge jump from field trials to commercial fields. But when a product’s value is obvious, farmers actually embrace new technology very quickly: both GMO crops and GPS achieved widespread adoption in a very short period of time.
Finally, technology developers should keep in mind that problems can be solved by old or simple technology. When people think about controlled-environment farming, their minds jump to fancy things like vertical farming — but with irrigation and mulch films, you’re 90% of the way there. Simply by adding a mulch film to heat the soil, farmers can greatly extend the growing season in northern climates by a month. This approach allowed us us to substantially increase the yield from our corn fields in Wisconsin.
This conversation illustrates a clear need for change in three key areas:
Federal funding for agricultural R&D
Given the unreliability of private market funding for agricultural biotechnology R&D, which often entails long turnaround times and low margins relative to traditional tech companies, substantial federal funding through research programs such as AgARDA is vital for accelerating R&D. AgARDA, based on the ARPA Advanced Research Projects Agency model, would allow the USDA to support the development of transformative technologies for focus areas of its choosing. However, despite its popularity, AgARDA, which was first authorized in the 2018 Farm Bill for $50 million annually for FY2019-2023, only received $2m in that timeframe. The USDA requested $5m for AgARDA in FY2022 and again in FY2023; it only received $1m each year. By contrast, ARPA-H, the human health equivalent, was authorized in FY2022 and immediately received its full $1 billion authorization, followed by $1.5b in FY2023.
The USDA has published an implementation framework for AgARDA. Unfortunately, misalignment between USDA and Congress appears to be preventing AgARDA from being fully funded to its authorized levels. Members of the Congressional agriculture committees want the USDA to show that it has made progress with the $2m it has received before they allocate additional funding, namely the appointment of a dedicated director and initiation of a pilot program with calls for grant proposals. However, the USDA has deemed the $2m insufficient to support long-term staff or a formal grant program, especially since the appropriations require annual renewal. The current impasse means that no AgARDA projects have been rolled out, despite the pressing nature of the research priorities identified by the USDA.
The following steps should be taken for AgARDA to achieve its full potential:
- USDA should produce a formal report to Congress of how it utilized the $2m thus far allocated.
- Congress should ensure that the bill to expand AgARDA authorization from $50m to $100m is passed as part of the upcoming Farm Bill.
- USDA should request, and Congress should approve, funding up to its full authorization for AgARDA in its FY2026 budget. Additionally, the appropriations should be granted on a three-year basis, like ARPA-H’s appropriations, to permit greater runway.
Regulatory oversight
The U.S. regulatory system for biotechnology needs to be a) expanded, with funding for a larger agency staff to process applications quickly; b) updated, to be flexible such that it can accommodate new-to-market technologies; and c) coordinated, to streamline approval processes.
The National Security Commission on Emerging Biotechnology (NSCEB) addresses these unmet needs in its interim report. First, NSCEB is “considering options to facilitate higher staffing levels”; this should be made a priority.
Second, concerning regulatory oversight, NSCEB identified three potential paths for improvement:
- discrete changes to individual statutes to reduce redundancies and gaps in biotechnology oversight;
- a single, unified regulatory process to assess any novel risks associated with biotechnology products relative to their conventional counterparts; and
- a hybrid approach that legislatively mandates coordination while facilitating individual agency review and risk assessment.
Of these, the hybrid approach would likely provide the greatest flexibility. In contrast, discrete changes to individual statutes will likely involve slow, piecemeal changes that can easily become outdated again. While a unified regulatory process may be more streamlined, the report’s phrasing creates a sharp binary delineation between biotech and conventional that does not reflect reality. Such a delineation could engender a lot of wasted time debating biotech versus conventional classification for a given product.
Finally, to address intra- and interagency coordination, the NSCEB presented two Farm Bill amendments that deserve Congressional support: the Biotechnology Oversight Coordination Act and the Agriculture Biotechnology Coordination Act.
Bioliteracy and agricultural education
Market demand and regulations are informed by consumer perceptions, which then impact R&D decisions. For example, fear of consumer and regulatory backlash can dissuade companies from investing in new genetic engineering technology for developing new plant varieties, despite their potential to improve agricultural sustainability. Increased bioliteracy across the American public would help consumers, businesses, and policymakers alike better understand new biotechnologies and engage with the burgeoning bioeconomy. This is a need that the NSCEB has also highlighted. At the K-12 level, improvements could comprise updating science curriculums to include contemporary topics like gene editing, as well as amending civics curriculums to better explain the modern functions of regulatory agencies. In addition, agricultural education can be embedded into biology and earth science curriculums to reconnect the public at large with the realities faced by producers. Similar to computer science literacy improvements through standard setting and funding, bioliteracy can be improved through state-level education initiatives.
The Federation of American Scientists values diversity of thought and believes that a range of perspectives — informed by evidence — is essential for discourse on scientific and societal issues. Contributors allow us to foster a broader and more inclusive conversation. We encourage constructive discussion around the topics we care about.
BioNETWORK: The Internet of Distributed Biomanufacturing
Summary
The future of United States industrial growth resides in the establishment of biotechnology as a new pillar of industrial domestic manufacturing, thus enabling delivery of robust supply chains and revolutionary products such as materials, pharmaceuticals, food, energy. Traditional centralized manufacturing of the past is brittle, prone to disruption, and unable to deliver new products that leverage unique attributes of biology. Today, there exists the opportunity to develop the science, infrastructure, and workforce to establish the BioNETWORK to advance domestic distributed biomanufacturing, strengthen U.S.-based supply chain intermediaries, provide workforce development for underserved communities, and achieve our own global independence and viability in biomanufacturing. Implementing the BioNETWORK to create an end-to-end distributed biomanufacturing platform will fulfill the Executive Order on Advancing Biotechnology and Biomanufacturing Innovation and White House Office of Science and Technology Policy (OSTP) Bold Goals for U.S. Biotechnology and Biomanufacturing.
Challenge and Opportunity
Biotechnology harnesses the power of biology to create new services and products, and the economic activity derived from biotechnology and biomanufacturing is referred to as the bioeconomy. Today, biomanufacturing and most other traditional non-biomanufacturing is centralized. Traditional manufacturing is brittle, does not enhance national economic impact or best use national raw materials/resources, and does not maximize innovation enabled by the unique workforce distributed across the United States. Moreover, in this era of supply chain disruptions due to international competition, climate change, and pandemic-sized threats (both known and unknown), centralized approaches that constitute a single point of attack/failure and necessarily restricted, localized economic impact are themselves a huge risk. While federal government support for biotechnology has increased with recent executive orders and policy papers, the overarching concepts are broad, do not provide actionable steps for the private sector to respond to, and do not provide the proper organization and goals that would drive outcomes of real manufacturing, resulting in processes or products that directly impact consumers. A new program must be developed with clear milestones and deliverables to address the main challenges of biomanufacturing. Centralized biomanufacturing is less secure and does not deliver on the full potential of biotechnology because it is:
- Reliant on a narrow set of feedstocks and reagents that are not local, introducing supply chain vulnerabilities that can halt bioproduction in its earliest steps of manufacturing.
- Inflexible for determining the most effective, stable, scalable, and safe methods of biomanufacturing needed for multiple products in large facilities.
- Serial in scheduling, which introduces large delays in production and limits capacity and product diversity.
- Bespoke and not easily replicated when it comes to selection and design of microbial strains, cell free systems, and sequences of known function outside of the facility that made them. Scale-up and reproducibility of biomanufacturing products are limited.
- Creating waste streams because circular economies are not leveraged.
- Vulnerable to personnel shortages due to shifting economic, health, or other circumstances related to undertraining of a biotechnology specialized workforce.
Single point failures in centralized manufacturing are a root cause of product disruptions and are highlighted by current events. The COVID-19 pandemic revealed that point failures in the workforce or raw materials created disruptions in the centralized manufacturing, and availability of hand sanitizers, rubber gloves, masks, basic medicines, and active pharmaceutical ingredients impacted every American. International conflict with China and other adversarial countries has also created vulnerabilities in the sole source access to rare earth metals used in electronics, batteries, and displays, driving the need for alternate options for manufacturing that do not rely on single points of supply. To offset this situation, the United States has access to workforce, raw materials, and waste streams geographically distributed across the country that can be harnessed by biomanufacturing to produce both health and industrial products needed by U.S. consumers. However, currently there are only limited distributed manufacturing infrastructure development efforts to locally process those raw materials, leaving societal, economic, and unrealized national security risks on the table. Nation-scale parallel production in multiple facilities is needed to robustly create products to meet consumer demand in health, industrial, energy, and food markets.
The BioNETWORK inverts the problem of a traditional centralized biomanufacturing facility and expertise paradigm by delivering a decentralized, resilient network enabling members to rapidly access manufacturing facilities, expertise, and data repositories, as needed and wherever they reside within the system, by integrating the substantial existing U.S. bioindustrial capabilities and resources to maximize nationwide outcomes. The BioNETWORK should be constructed as an aggregate of industrial, academic, financial, and nonprofit entities, organized in six regionally-aligned nodes (see figure below for notional regional distribution) of biomanufacturing infrastructure that together form a hub network that cultivates collaboration, rapid technology advances, and workforce development in underserved communities. The BioNETWORK’s fundamental design and construction aligns with the need for new regional technology development initiatives that expand the geographical distribution of innovative activity in the U.S., as stated in the CHIPS and Science Act. The BioNETWORK acts as the physical and information layer of manufacturing innovation, generating market forces, and leveraging ubiquitous data capture and feedback loops to accelerate innovation and scale-up necessary for full-scale production of novel biomaterials, polymers, small molecules, or microbes themselves. As a secure network, BioNETWORK serves as the physical and virtual backbone of the constituent biomanufacturing entities and their customers, providing unified, distributed manufacturing facilities, digital infrastructure to securely and efficiently exchange information/datasets, and enabling automated process development. Together the nodes function in an integrated way to adaptively solve biotechnology infrastructure challenges as well as load balancing supply chain constraints in real-time depending on the need. This includes automated infrastructure provisioning of small, medium, or large biomanufacturing facilities, supply of regional raw materials, customization of process flow across the network, allocation of labor, and optimization of the economic effectiveness. The BioNETWORK also supports the implementation of a national, multi-tenant cloud lab and enables a systematic assessment of supply chain capabilities/vulnerabilities for biomanufacturing.
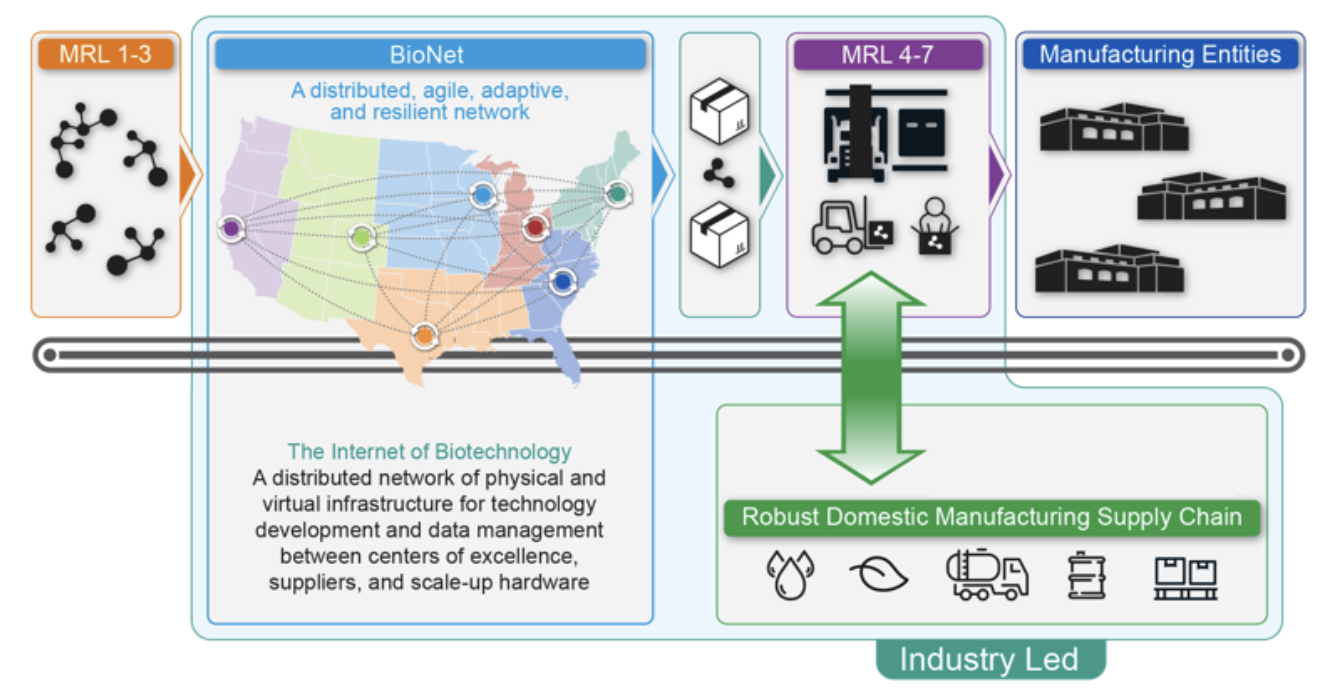
As a secure network, BioNETWORK serves as the physical and virtual backbone of the constituent biomanufacturing entities and their customers, providing unified, distributed manufacturing facilities, digital infrastructure to securely and efficiently exchange information/datasets, and enabling automated process development.
Plan of Action
Congress should appropriate funding for an interagency coordination office co-chaired by the OSTP and the Department of Commerce (DOC) and provide $500 million to the DOC, Department of Energy (DOE), and Department of Defense (DOD) to initiate the BioNETWORK and use its structure to fulfill economic goals and create industrial growth opportunities within its three themes:
- Provide alternative supply chain pathways via biotechnologies and biomanufacturing to promote economic security. Leverage BioNETWORK R&D opportunities to develop innovative biomanufacturing pathways that could address supply chain bottlenecks for critical drugs, chemicals, and other materials.
- Explore distributed biomanufacturing innovation to enhance supply chain resilience. Leverage BioNETWORK R&D efforts to advance flexible and adaptive biomanufacturing platforms to mitigate the effects of supply chain disruptions.
- Address standards and data infrastructure to support biotechnology and biomanufacturing commercialization and trade. Leverage BioNETWORK R&D needed to enable data interoperability across the network to enable scale-up and increase global competitiveness.
To achieve these goals, the policy Plan of Action includes the following steps:
1. Congress should appropriate $10 million to establish an interagency coordination office within OSTP that is co-chaired by the DOC. This fulfills the White House Executive Order and CHIPs and Science mandates for better interagency coordination among the DOE, DOC, DOD, National Institute of Standards and Technology (NIST), and the National Science Foundation (NSF).
2. Congress should then appropriate $500 million to DOC and DOE to fund a biomanufacturing moonshot that includes creating the pilot network of three nodes to form the BioNETWORK in regions of the U.S. within six months of receiving funding. This funding should be managed by the interagency coordination office in collaboration with a not-for-profit organization whose mission is to build, deploy, and manage the BioNETWORK together with the federal entities. The role of the not-for-profit is to ensure that a trusted, unbiased partner (not influenced by outside entities) is involved, such that the interests of the taxpayer, U.S. government, and commercial sectors are all represented in the most beneficial way possible. The mission should include education, workforce development, safety/security, and sustainment as core principles, such that the BioNETWORK can stand alone once established. The new work to build the network should also synergize with the foundational science of the NSF and the national security focus of DOD biotechnology programs.
3. Continued investment of an additional $500 million should be appropriated by Congress to create economic incentives to sustain and transition the BioNETWORK from public funding to full commercial operation. This step requires evaluation of concrete go/no-go milestones and deliverables to ensure on-time, on-budget operations have been met. The interagency coordination office should work with DOC, DOE, DOD, and other agencies to leverage these incentives and create other opportunities to promote the BioNETWORK so that it does not require public funding to keep itself sustainable and can obtain private funding.
Create a Pilot Network of Three Nodes
To accelerate beyond current biomanufacturing programs and efforts, the first three nodes of the BioNETWORK should be constructed in three new disparate geographic regions (i.e., East, Midwest, West, or other locations with relevant feedstocks, workforce, or component infrastructure) to show the networking capabilities for distributed manufacturing. The scale of funding required to design, construct, and deploy the first three nodes is $500 million. The initiation and construction of the BioNETWORK should commence within six months. The DOE should lead the initiation and deployment of the technical construction of the BioNETWORK through Theme 2 of their Biomanufacturing goals, which “seeks alternative processes to produce chemicals and materials from renewable biomass and intermediate feedstocks by developing low-carbon-intensity product pathways and promoting a circular economy for materials.” Each node should create regional partnerships that have four entities (a physical manufacturing facility, a cell programming entity, an academic research and development entity, and a workforce/resource entity). All four entities will contain both physical facilities such as industrial fermentation and wet lab space, as well as the workforce needed to run them. On top of the pilot nodes, a science and technology/engineering integrator of the system should be identified to coordinate the effort and lead security/safety efforts for the physical network. Construction of the initial BioNETWORK should be completed within two years.
Achievement of the BioNETWORK goals requires the design plan to:
- Leverage and use regional feedstocks and reagents across the U.S. as inputs to bioproduction to create robustness in the earliest steps of manufacturing.
- Automate the integrated use of small, intermediate, and large-scale biomanufacturing facilities so that they are effective, stable, scalable, and safe for biomanufacturing demand.
- Parallelize scheduling of infrastructure and resources to minimize delays in production and maximize capacity and product diversity.
- Incorporate methods for replication when it comes to selection and design of microbial strains, cell free systems, and sequences of known function.
- Reuse waste streams to create circular economies.
- Include infrastructure biomanufacturing standards from NIST.
The BioNETWORK construction milestones should fulfill the White House OSTP bold goals through new capabilities delivered via distributed manufacturing infrastructure:
- Networked data for distributed biomanufacturing—“establishing a Data Initiative to ensure that high-quality, wide-ranging, easily accessible, and secure biological data sets can drive breakthroughs for the U.S. bioeconomy.”
- Domestic distributed biomanufacturing infrastructure—“expanding domestic capacity to manufacture all the biotechnology products we invent in the United States and support a resilient supply chain.”
- Local hubs for workforce development—“growing training and education opportunities for the biotechnology and biomanufacturing workforce of the future.”
Full Network: Plan for Sustainability
Congress and executive branch agencies establish economic incentives for commercial entities, state/local governments, and consumers of bioindustrial manufacturing products to create commercialization pathways that enhance local economies while also supporting the national network. These include tax credits, tax breaks, low interest loans, and underwritten loans as a starting point. To facilitate tech transition, unique lab-to-market mechanisms and proven tools to address market failure and applied technologies gaps should be used in conjunction with those in the Inflation Reduction Act. This includes prize and challenge competitions, market shaping procurement or loan programs, and streamlined funding of open, cross-disciplinary research, and funding at the state and local levels.
A new public-private partnership could coordinate across multiple efforts to ensure they drive toward rapid technology deployment and integration. This includes implementing a convertible debt plan that rewards BioNETWORK members with equity after reaching key milestones, providing an opportunity for discounted buyout by other investors during rounds of funding, and working with the federal government to design market-shaping mechanisms such as advance market commitments to guarantee purchase of a bioproduction company’s spec-meeting product.
Additionally, the BioNETWORK should be required to expand the repertoire of domestic renewable raw materials into a suite of high-demand, industry-ready products as prescribed in the DOC’s goals in biomanufacturing. This will ensure all regions have support for commercial goods and can automatically assess domestic supply chain capabilities and vulnerabilities, and are provided compensatory remediation on demand. The full BioNETWORK consists of six nodes—aligned to each of the major geographic regions and/or EDA regions in the United States—which have unique raw materials, workforce, infrastructure, and consumption of products that contribute to supporting the overall network functionality. The full BioNETWORK should be active within five years of project initiation and be evaluated against phased milestones throughout.
Conclusion
Networked solutions are resilient and enduring. A single factory is at risk of transfer to foreign ownership, closure, or obsolescence. The BioNETWORK creates connectivity among distributed biomanufacturing physical infrastructure to form a network with a robust domestic value chain. Today’s biomanufacturing investments suffer from the need to vertically integrate due to lack of flexible capacity across the value chain, which raises capital requirements and overall risk. The BioNETWORK drives horizontal integration through the network nodes via new infrastructure, connecting physical infrastructure of the nodes within the system. The result is a multi-sided marketplace for biotechnology innovation, products, and commercialization.
The federal government should initiate a new program and select performers within the next six months to begin the research, development, and construction of the first three nodes of the BioNETWORK. Taking action to establish the BioNETWORK ensures that the United States has the necessary physical and virtual infrastructure to grow the bioeconomy and its international leadership in biotechnology. The BioNETWORK creates new job opportunities for people across the country where training in biotechnology expands the skill sets of people with broad-spectrum applicability from trades to advanced degrees. The BioNETWORK drives circular economies where raw materials from rural and urban centers enter the network and are transformed into high-value products such as advanced materials, pharmaceuticals, food, and energy. The BioNETWORK protects U.S. supply chain resiliency through distributed manufacturing and links regional development into a national capability to establish biomanufacturing as a pillar of economic and technological growth for today and into the 22nd century.
Establishment of the BioNETWORK scales, connects, and networks the impact of a hub and tailors it to the needs of bioindustrial manufacturing, which requires regional feedstocks and integration of small-, intermediate-, and large-scale industrial fermentation facilities scattered across the United States to form an end-to-end distributed biomanufacturing platform. Similar to the goals of the EDA hub program, the BioNETWORK will accelerate regional economic activity, workforce development, and re-establishment of domestic manufacturing. Leveraging activity of the EDA and NSF Biofoundries program is an opportunity for coordination across the interagency.
Retrofitting existing small-, intermediate-, and large-scale biomanufacturing facilities/plants is necessary to construct the connected BioNETWORK. This includes new/modified fermentation equipment, scale-up and purification hardware, software/communications for networking, transportation, load-balancing, and security infrastructure.
Clear, measurable intermediate milestones and deliverables are required to ensure that the BioNETWORK is on track. Every three months, key performance metrics and indicators should be used to demonstrate technical functionality. Planned economic and workforce targets should be established every year and tracked for performance. Adjustments to the technical and business plans should be implemented if needed to ensure the overarching goals are achieved.
A major outcome of the BioNETWORK program is that biomanufacturing in the United States becomes on par with the other traditional pillars of manufacturing such as chemicals, food, and electronics. Workforce retraining to support this industry leads to new high-paying jobs as well as new consumer product sectors and markets with new avenues for economic growth. Failure to deploy the BioNETWORK leaves the United States vulnerable to supply chain disruption, little to no growth in manufacturing, and out competition by China and other peer nations that are investing in and growing biotechnology.
Secondary milestones include key performance indicators, including increased capacity, decrease in production time, robustness (more up time vs. down time), cheaper costs, better use of regional raw materials, etc.
“The US needs to lean into an old strength”: Maintaining Progress and Growing US Biomanufacturing
The U.S. bioeconomy has been surging forward, charged by the Presidential Executive Order 14081 and the CHIPS and Science Act. However, there are many difficult challenges that lay ahead for the U.S. bioeconomy, including for U.S. biomanufacturing capabilities. U.S. biomanufacturing has been grappling with issues in fermentation capacity including challenges related to scale-up, inconsistent supply chains, and downstream processing. While the U.S. government works on shoring up these roadblocks, it will be important to bring industry perspectives into the conversation to craft solutions that not only addresses the current set of issues but looks to mitigate challenges that may arise in the future.
To get a better understanding of industry perspectives on the U.S. bioeconomy and the U.S. biomanufacturing sector, the Federation of American Scientists interviewed Dr. Sarah Richardson, the CEO of MicroByre. MicroByre is a climate-focused biotech startup that specializes in providing specialized bacteria based on the specific fermentation needs of its clients. Dr. Richardson received her B.S. in biology from the University of Maryland in 2004 and a Ph.D. in human genetics and molecular biology from Johns Hopkins University School of Medicine in 2011. Her extensive training in computational and molecular biology has given her a unique perspective regarding emerging technologies enabled by synthetic biology.
FAS: The U.S. Government is focused on increasing fermentation capacity, including scale-up, and creating a resilient supply chain. In your opinion, are there specific areas in the supply chain and in scale-up that need more attention?
Dr. Sarah Richardson: The pandemic had such an impact on supply chains that everyone is reevaluating the centralization of critical manufacturing. The United States got the CHIPS and Science Act to invest in domestic semiconductor manufacturing. The voting public realized that almost every need they had required circuits. Shortages in pharmaceuticals are slowly raising awareness of chemical and biomedical manufacturing vulnerabilities as well. The public has even less insight into vulnerabilities in industrial biomanufacturing, so it is important that our elected officials are proactive with things like Executive Order 14081.
When we talk about supply chains we usually mean the sourcing and transfer of raw, intermediate, and finished materials — the flow of goods. We achieve robustness by having alternative suppliers, stockpiles, and exacting resource management. For biomanufacturing, an oft raised supply chain concern is feedstock. I can and will expound on this, but securing a supply of corn sugar is not the right long-term play here. Shoring up corn sugar supplies will not have a meaningful impact on industrial biomanufacturing and should be prioritized in that light.
Biomanufacturing efforts are different from the long standing production of consumer goods in that they are heavily tied to a scientific vendor market. As we scale to production, part of our supply chain is a lot of sterile plastic disposable consumables. We compete with biomedical sectors for those, for personal protective equipment, and for other appliances. This supply chain issue squeezed not just biomanufacturing, but scientific research in general.
We need something that isn’t always thought of as part of the supply chain: specialized infrastructural hardware. This may not be manufactured domestically. Access to scale up fermentation vessels is already squeezed. The other problem is that no matter where you build them, these vessels are designed for the deployment of canonical feedstocks and yeasts. Addressing the manufacturing locale would offer us the chance to innovate in vessel and process design and support the kinds of novel fermentations on alternate feedstocks that are needed to advance industrial biomanufacturing. There are righteous calls for the construction of new pilot plants. We should make sure that we take the opportunity to build for the right future.
One of the indisputable strengths of biomanufacturing is the potential for decentralization! Look at microbrewing: fermentation can happen anywhere without country-spanning feedstock pipelines. As we onboard overlooked feedstocks, it may only be practical to leverage them if some fermentation happens locally. As we look at supply chains and scale up we should model what that might look like for manufacturing, feedstock supply chains, and downstream processing. Not just at a national level, but at regional and local scales as well.
There are a lot of immediate policy needs for the bioeconomy, many of which are outlined in Executive Order 14081. How should these immediate needs be balanced with long-term needs? Is there a trade-off?
Counterintuitively, the most immediate needs will have the most distant payoffs! The tradeoff is that we can’t have every single detail nailed down before work begins. We will have to build tactically for strategic flexibility. Climate change and manufacturing robustness are life or death problems. We need to be open to more creative solutions in funding methods, timeline expectations; in who comes to the table, in who around the table is given the power to affect change, and in messaging! The comfortable, familiar, traditional modes of action and funding have failed to accelerate our response to this crisis.
We have to get started on regulation yesterday, because the only thing that moves slower than technology is policy. We need to agree on meaningful, aggressive, and potentially unflattering metrics to measure progress and compliance. We need to define our terms clearly: what is “bio-based,” does it not have petroleum in it at all? What does “plant-based” mean? What percentage of a product has to be renewable to be labeled so? If it comes from renewable sources but its end-of-life is not circularizable, can we still call it “green”?
We need incentives for innovation and development that do not entrench a comfortable but unproductive status quo. We need to offer stability to innovators by looking ahead and proactively incubating the standards and regulations that will support safety, security, and intellectual property protection. We should evaluate existing standards and practices for inflexibility: if they only support the current technology and a tradition that has failed to deliver change, they will continue to deliver nothing new as a solution.
We need to get on good footing with workforce development, as well. A truly multidisciplinary effort is critical and will take a while to pull off; it takes at least a decade to turn a high school student into a scientist. I only know of one national graduate fellowship that actually requires awardees to train seriously in more than one discipline. Siloing is a major problem in higher education and therefore in biomanufacturing. What passes for “multidisciplinary” is frequently “I am a computer scientist who is not rude to biologists” or “our company has both a chemical division and an AI division.” A cross-discipline “bilingual” workforce is absolutely critical to reuniting the skill sets needed to advance the bioeconomy. Organizations like BioMADE with serious commitments to developing a biomanufacturing workforce cannot effectively address the educational pipeline without significantly more support.
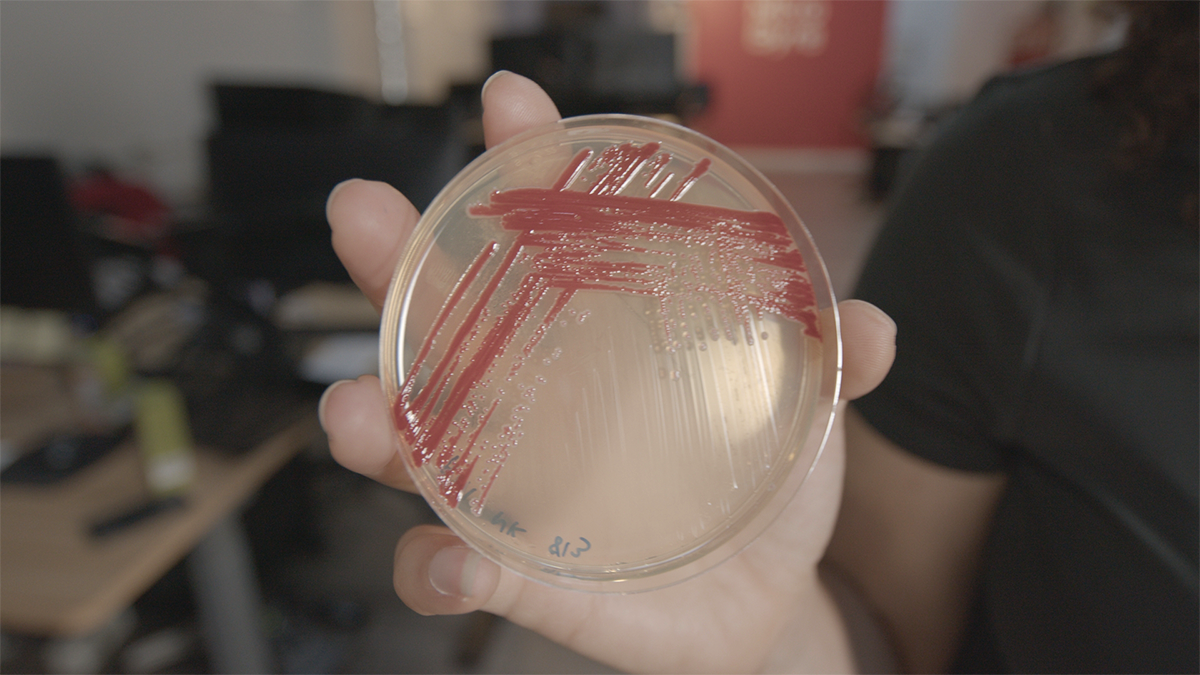
MicroByre is working to advance alternatives to substrates currently favored by the bioeconomy.
When we emphasize the collection of data — which data are we talking about? Is the data we have collected already a useful jumping off point for what comes next? Are the models relevant for foreseeable changes in technology, regulation, and deployment? For some of it, absolutely not. As every responsible machine learning expert can tell you, data is not something you want to skimp or cheap out on collecting or curating. We have to be deliberate about what we collect, and why. Biases cannot all be avoided, but we have to take a beat to evaluate whether extant models, architecture, and sources are relevant, useful, or adaptable. A data model is as subject to a sunk cost fallacy as anything else. There will be pressure to leverage familiar models and excuses made about the need for speed and the utility of transfer learning. We cannot let volume or nostalgia keep us from taking a sober look at the data and models we currently have, and which ones we actually need to get.
What are the major pain points the biomanufacturing industry is currently facing?
Downstream processing is the work of separating target molecules from the background noise of production. In purely chemical and petrochemical fields, separation processes are well established, extensively characterized, and relatively standardized. This is not the case in industrial biomanufacturing, where upstream flows are arguably more variable and complex than in petrochemicals. Producers on the biomedical side of biomanufacturing who make antibiotics, biologics, and other pharmaceuticals have worked on this problem for a long time. Their products tend to be more expensive and worth specialized handling. The time the field has spent developing the techniques in the urgent pursuit of human health works in their favor for innovation. However, separating fermentation broth from arbitrary commodity molecules is still a major hurdle for a bioindustrial sector already facing so many other simultaneous challenges. Without a robust library of downstream processing methods and a workforce versant in their development and deployment, new industrial products are viewed as significant scaling risks and are funded accordingly.
There is fatigue as well. For the sake of argument, let us peg the onset of the modern era of industrial biomanufacturing to the turn of the latest century. There have been the requisite amount of promises any field must make to build itself into prominence, but there has not been the progress that engenders trust in those or future promises. We have touted synthetic biology as the answer for two and a half decades but our dependence on petroleum for chemicals is as intense as ever. The goodwill we need to shift an entire industry is not a renewable resource. It takes capital, it takes time, and it takes faith that those investments will pay off. But now the chemical companies we need to adopt new solutions have lost some confidence. The policy makers we need to lean into alternative paths and visionary funding are losing trust. If the public from whence government funding ultimately springs descends into skepticism, we may lose our chance to pivot and deliver.
The right investment right now will spell the difference between life and death on this planet for billions of people.
This dangerous dearth of confidence can be addressed by doing something difficult: owning up to it. No one has ever said “oh goody — a chance to do a postmortem!”. But such introspective exercises are critical to making effective changes. A lack of reflection is a tacit vote for the status quo, which is comfortable because we’re rarely punished for a lack of advocacy. We should commission an honest look at the last thirty years — without judgment, without anger, and without the need to reframe disappointing attempts as fractional successes for granting agencies, or position singular successes as broadly representative of progress for egos.
Biomanufacturing is so promising! With proper care and attention it will be incredibly transformative. The right investment right now will spell the difference between life and death on this planet for billions of people. We owe it to ourselves and to science to do it right — which we can only do by acknowledging what we need to change and then truly committing to those changes.
Corn sugar tends to be the most utilized biomass in the bioeconomy. What are the issues the U.S. faces if it continues to rely solely on corn sugar as biomass?
History shows that low-volume, high-margin fine chemicals can be made profitable on corn sugar, but high-volume, low-margin commodity chemicals cannot. Projects that produce fine chemicals and pharmaceuticals see commercial success but suffer from feedstock availability and scaling capacity. Success in high-margin markets encourages people to use the exact same technology to attempt low-margin markets, but then they struggle to reduce costs and improve titers. When a commodity chemical endeavor starts to flag, it can pivot to high-margin markets. This is a pattern we see again and again. As long as corn sugar is the default biomass, it will not change; the United States will not be able to replace petrochemicals with biomanufacturing because the price of corn sugar is too high and cannot be technologically reduced. This pattern is also perpetuated because the yeast we usually ask to do biomanufacturing cannot be made to consume anything but corn sugar. We also struggle to produce arbitrary chemicals in scalable amounts from corn sugar. We are stuck in an unproductive reinforcing spiral.
Even if commodity projects could profit using corn sugar, there is not enough to go around. How much corn sugar would we have to use to replace even a fifth of the volume of petroleum commodity chemicals we currently rely on? How much more land, nitrogen, water, and additional carbon emissions would be needed? Would chemical interests begin to overpower food, medical, and energy interests? What if a pathogen or natural disaster wiped out the corn crop for a year or two? Even if we could succeed at manufacturing commodities with corn sugar alone, locking out alternatives makes the United States supply chain brittle and vulnerable.
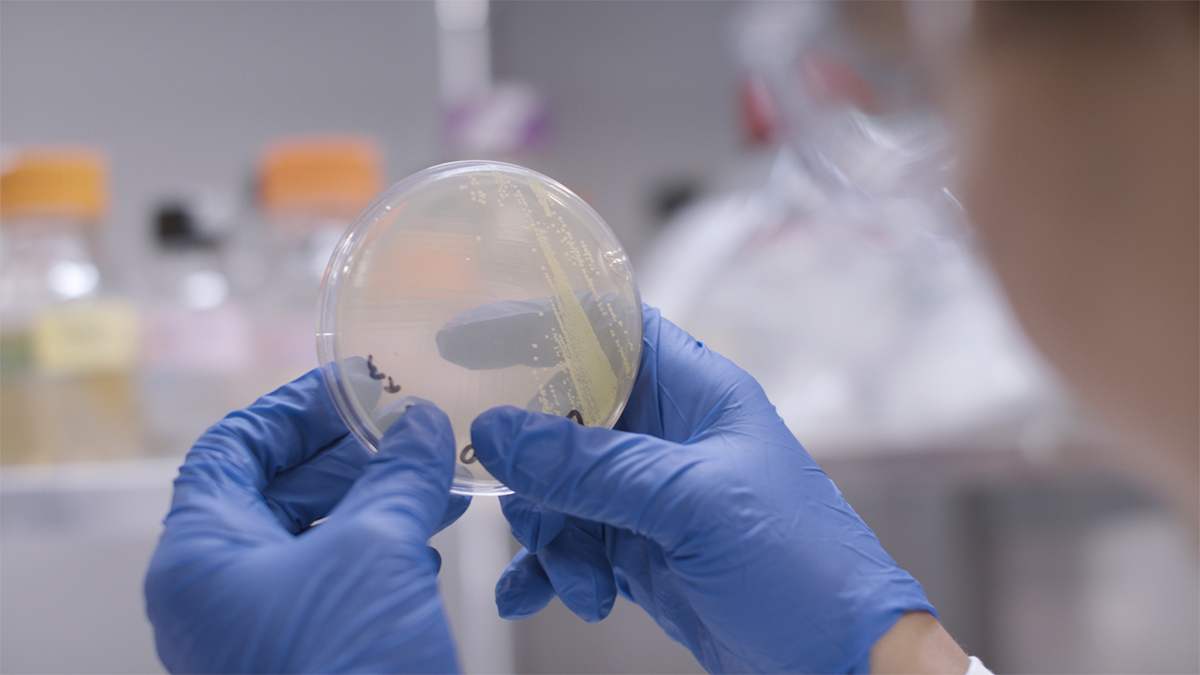
MicroByre is working to advance alternatives to substrates currently favored by the bioeconomy.
Continued reliance on corn sugar slows our technological development and stifles innovation. Specialists approaching manufacturing problems in their domain are necessarily forced to adopt the standards of neighboring domains. A chemical engineer is not going to work on separating a biomass into nutrition sources when no microbiologist is offering an organism to adopt it. A molecular biologist is not going to deploy a specialized metabolic pathway dependent on a nutrition source not found in corn sugar. Equipment vendors are not going to design tools at any scale that stray from a market demand overwhelmingly based on the use of corn sugar. Grantors direct funds with the guidance of universities and industry leaders, who are biased towards corn sugar because that’s what they use to generate quick prototypes and spin out new start up companies.
The result of relying on corn sugar is an entrenched field and consequently we might lose our chance to make a difference. Without introducing low-cost, abundant feedstocks like wastes, we run the risk of disqualifying an entire field of innovation.
What does the U.S. need to do in order for other biomass sources to be utilized beyond corn sugar? Are there ideas (or specific programs) that the U.S. government could supercharge?
Federal agencies must stop funding projects that propose to scale familiar yeasts on corn sugars to produce novel industrial chemicals. We must immediately stop funding biomass conversion projects meant to provide refined sugars to such endeavors. And we must stop any notion of dedicating arable land solely to corn sugar solely for the purposes of biomanufacturing new industrial products. The math does not and will not work out. The United States must stop throwing money and support at such things that seem like they ought to succeed any minute now, even though we have been waiting for that success for 50 years without any meaningful changes in the economic analysis or technology available.
Ironically, we need to take a page from the book that cemented petroleum and car supremacy in this country. We need to do the kind of inglorious, overlooked, and subsequently taken for granted survey of the kind that enabled the Eisenhower Interstate System to be built.
We need to characterize all of the non-corn feedstocks and their economic and microbial ecosystems. We need to know how much of each biomass exists, what it is composed of, and who is compiling where. We need to know what organisms rot it and what they produce from it. We need to make all of that data as freely available as possible to lower the barriers of entry for cross-disciplinary teams of researchers and innovators to design and build the logistical, microbiological, chemical, and mechanical infrastructure necessary. We need to prioritize and leverage the complex biomasses that cannot just be ground into yeast food.
We need to get the lay of the land so – to use the roadway analogy – we know where to pour the asphalt. An example of this sort of effort is the Materials Genome Initiative, which is a crosscutting multi-agency initiative for advancing materials and manufacturing technology. (And which has, to my chagrin, stolen the term “genome” for non-biological purposes.) An even more visible example to the public is a resource like the Plant Hardiness Zone Map that provides a basis for agricultural risk assessment to everyone in the country.
The United States needs to lean into an old strength and fund infrastructure that gives all the relevant specialties the ability to collaborate on truly divergent and innovative biomass efforts. The field of industrial biomanufacturing must make a concerted effort to critically examine a history of failed technical investments, shake off the chains of the status quo, and guide us into true innovation. Infrastructure is not the kind of project that yields an immediate return. If venture capital or philanthropy could do it, they would have already. The United States must flex its unique ability to work on a generational investment timeline; to spend money in the very short term on the right things so as to set everyone up for decades of wildly profitable success — and a safer and more livable planet.